RV Failure in COVID-19
The FLARE Four
- Right ventricular dysfunction commonly occurs in critical illness, particularly in patients with sepsis and ARDS, and is associated with increased risk of death
- Case series suggest that right ventricular failure can occur in COVID-19
- Diagnosis of right ventricle failure requires high clinical suspicion. While formal echocardiography may not be readily available during the SARS-CoV-2 pandemic, point-of-care ultrasound (with appropriate training), clinical exam, EKG and hemodynamic monitoring can be used to make the diagnosis
- To date, there is no convincing evidence that critically ill patients with SARS-CoV-2 are more prone to developing right ventricular dysfunction or failure than similar ICU patients without SARS-CoV-2
Many people are saying...COVID-19 may cause RV failure.
Subscribe to the latest updates from FLARE Advances in Motion
Introduction
Case series have described RV dysfunction in severe COVID-19, and postulated its association with COVID-19 may be a new phenomenon. The presence of RV dysfunction in acute respiratory distress syndrome (ARDS) has long been recognized (Vieillard-Baron et al. 2018), but its significance in SARS-CoV-2 infection has not yet been fully characterized (Li et al. 2020; Creel-Bulos et al. 2020). In tonight’s FLARE, we review the epidemiology and pathophysiology of critical illness-associated RV dysfunction to inform the management of patients suffering from severe COVID-19.
Physiology of the Pulmonary Circulation
Unlike the systemic circulation, the pulmonary circulation represents a low resistance, low pressure system - even during periods of high cardiac output (such as exercise or critical illness), during which recruitment of unfilled or underfilled vessels helps to maintain these parameters (Naeije and Chesler 2012). Although the pulmonary vasculature is not a simple ohmic resistor (due to the potential for vascular recruitment), Ohm’s law can be used to describe the relationship between resistance to flow, the pressure gradient across the vascular bed and the flow rate. In the case of the pulmonary circulation, pulmonary vascular resistance (PVR) is therefore defined as the difference between mean pulmonary pressure and pulmonary capillary wedge pressure (an estimate of the left atrial pressure) divided by the cardiac output:
PVR = (mPAP-PCWP) / CO
The structure of the RV differs significantly from that of the left ventricle (LV). The RV consists of a thin free wall and a more muscular interventricular septum (Figure 1) (C. Chan and Klinger 2015). The normal RV can generate a maximum systolic pressure of only approximately 50mmHg (McIntyre and Sasahara 1971; Vlahakes, Turley, and Hoffman 1981; Sutton, Hall, and Kerr 1977). In the setting of chronically increased afterload, however, the RV may hypertrophy and thereby generate pressures rivaling or exceeding systemic arterial pressures.
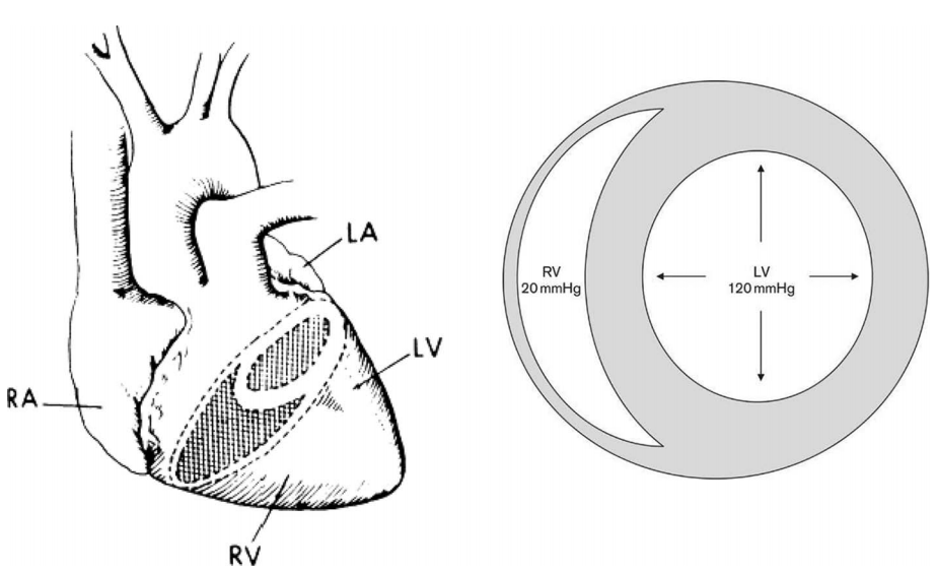
Figure 1
Structure and shape of the adult right and left ventricles (C. M. Chan and Klinger 2008).
Pathophysiology of RV Failure
Unlike the LV (which can tolerate large swings in afterload), the RV is very sensitive to even small changes in afterload. An untrained RV (i.e. without hypertrophy) suffers a significant decrease in contractility in the face of relatively small increases in mean pulmonary arterial pressure (Figure 2).
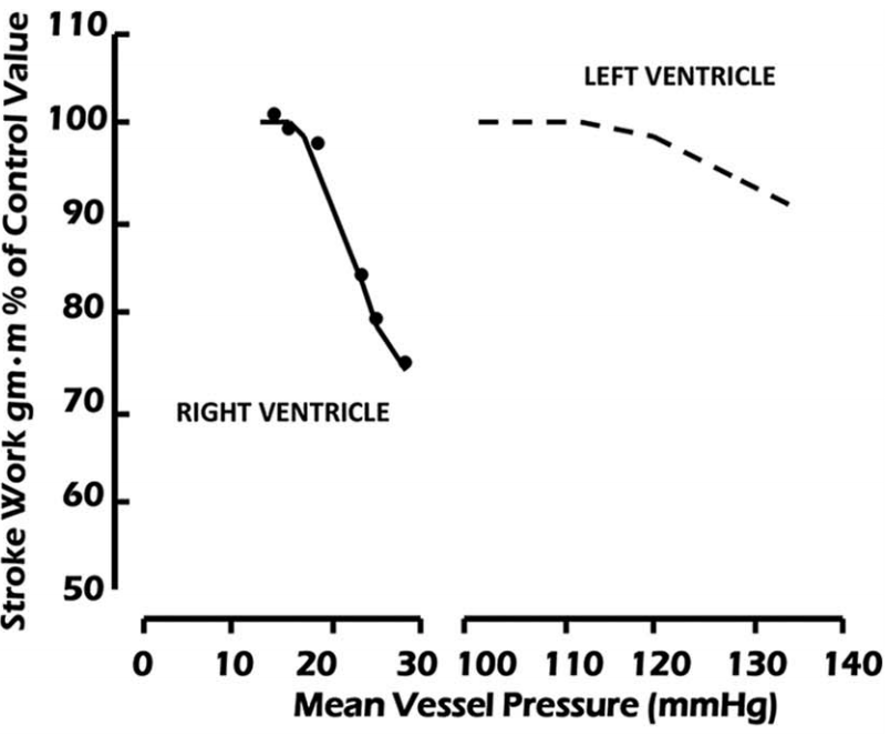
Figure 2
Comparison of stroke work versus mean aortic pressure in the left ventricle and mean pulmonary artery pressure in the right ventricle (both referred to as mean vessel pressure on x-axis) (C. Chan and Klinger 2015).
As RV afterload increases and RV output declines, the RV enlarges and dilates. Because RV free wall mobility is limited by the relatively non-compliant pericardium, RV dilation leads to compression of the RV and flattening of the interventricular septum. The flattened interventricular septum in turn impinges on the LV, impairing LV distensibility and function. These changes can be appreciated by echocardiogram, in which septal flattening should raise suspicion for increased RV volume and/or pressure (“D-sign” seen in Figure 3) (Ventetuolo and Klinger 2014).
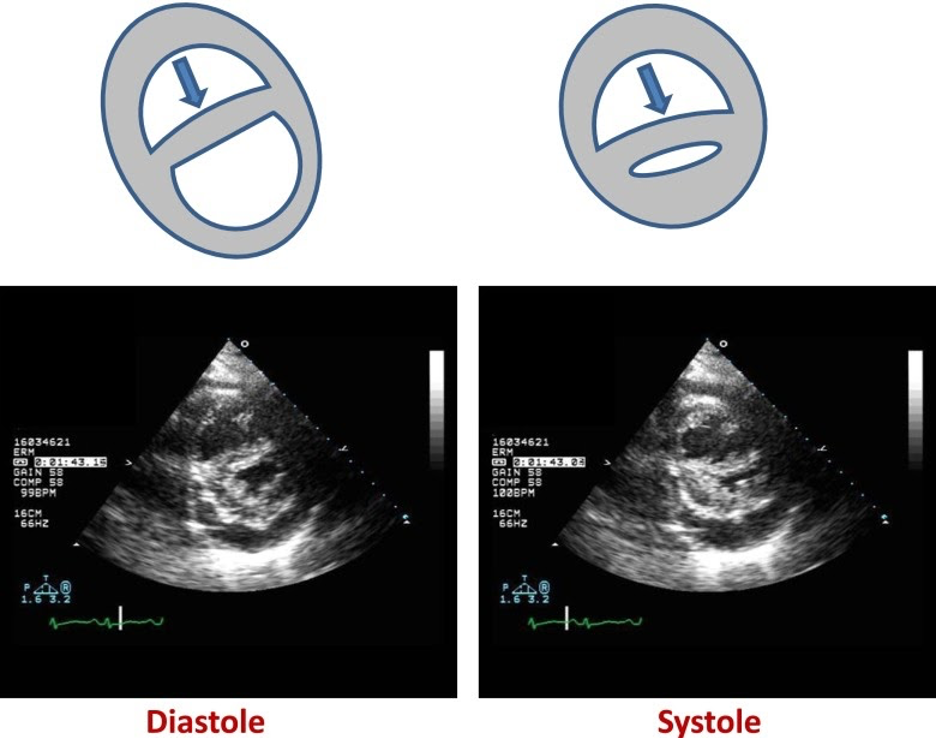
Figure 3
The position of the interventricular septum during the cardiac cycle is determined by the difference between RV and LV pressure. Under normal conditions, LV end-diastolic pressure is greater than RV end-diastolic pressure, and the septum bows toward the RV during diastole. As the RV fails, RV end-diastolic pressure begins to exceed that of the left ventricle (LV) and the septum bows toward the LV during diastole forming a “D”-shaped pattern and impaired LV filling (left panel). When RV failure occurs due to elevated pulmonary vascular resistance, the combination of high RV systolic pressure and decreased LV filling may lead to near obliteration of the LV at end systole (right panel)
(Ventetuolo and Klinger 2014).
While the above sequence of events alone would result in hemodynamic compromise, ischemia also plays a significant role in RV dysfunction (Vlahakes, Turley, and Hoffman 1981). In the healthy heart, the RV receives relatively equal blood flow during both systole and diastole, since at no point during the cardiac cycle is the right ventricular pressure greater than the aortic root pressure. However, as systemic hypotension develops and/or as RV pressure increases, systolic coronary filling is impaired and RV oxygen supply fails to keep pace with demand (van Wolferen et al. 2008) (Figure 4). This further worsens RV function and output, causing further volume and pressure overload, and resulting in worsening RV impingement upon the LV and worsening systemic hypotension. This systemic hypotension decreases the coronary perfusion pressure further, exacerbating RV ischemia, and ultimately results in a catastrophic cascade of events, dramatically referred to as the “RV death spiral” (Figure 5).
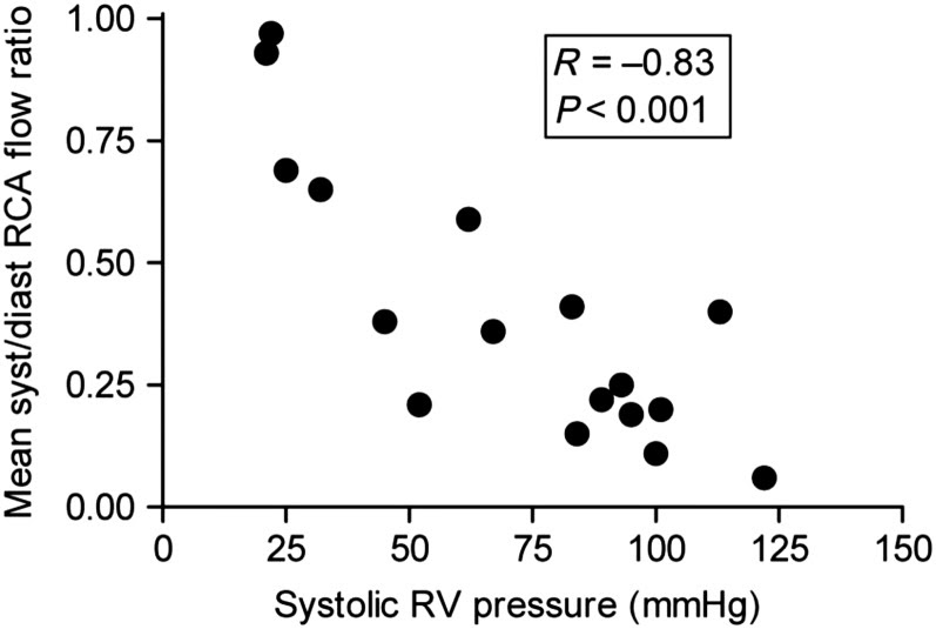
Figure 4
RCA mean systolic/diastolic flow ratio is plotted against right ventricular systolic pressure (RVSP). Note that as pressure increases in the right ventricle, coronary perfusion of the right heart falls (van Wolferen et al. 2008).
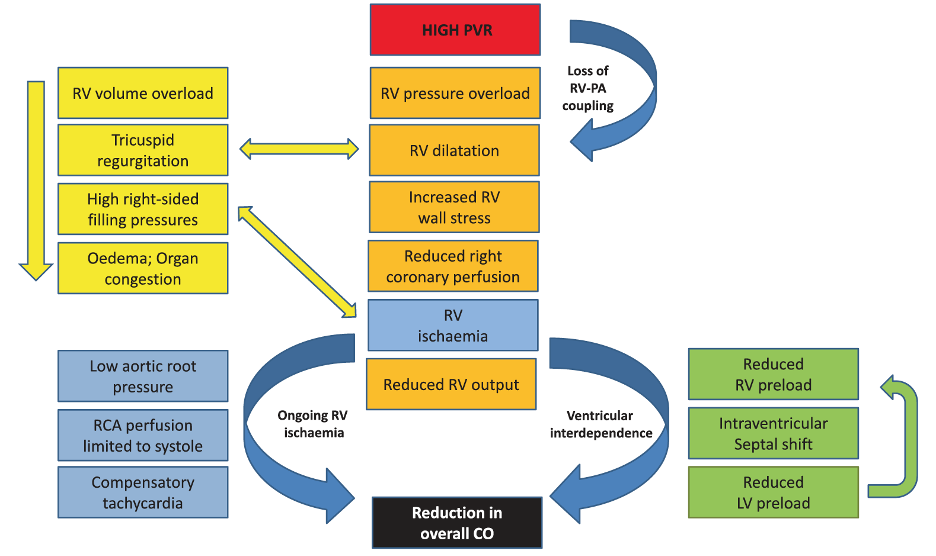
Figure 5
The “RV death spiral.” Increased RV afterload leads to RV dilatation, reduced cardiac output, and decreased right coronary perfusion, which further worsens RV dysfunction and perpetuates the cycle (Price et al. 2010).
RV Failure in the ICU
RV dysfunction is common in critically ill patients. The reported incidence of RV dysfunction varies widely between studies but is estimated to occur in 25-50% of patients with ARDS (Zochios et al. 2017). This variability likely reflects differences in study population and in diagnostic criteria. Several studies have also demonstrated an association between RV dysfunction, or increase in pulmonary vascular resistance, and increased mortality, particularly in patients with more severe abnormalities (Boissier et al. 2013; Bull et al. 2010; Mekontso Dessap et al. 2016). It is unclear if the increase in mortality is a result of RV dysfunction or if the presence of RV dysfunction reflects the severity of underlying illness. For example, RV dysfunction appears to be associated with higher driving pressures and need for prone ventilation, suggesting that increased RV dysfunction is related to worse lung injury (Boissier et al. 2013; Lhéritier et al. 2013).
The reasons why patients in the ICU are prone to developing RV dysfunction can be broadly grouped into two categories: (1) factors that cause primary myocardial depression (which may affect both ventricles) and (2) factors that increase RV afterload.
Direct Myocardial Depression
Some of the RV dysfunction that occurs in the ICU is secondary to primary myocardial dysfunction related to increased levels of pro-inflammatory cytokines or by direct invasion of cardiac myocytes by viruses (C. M. Chan and Klinger 2008; Kadokami et al. 2001; Bowles et al. 2003). As reviewed in a prior FLARE, a viral myocarditis has been proposed as a possible mechanism for SARS-CoV-2-associated myocardial dysfunction. At that time, there was a paucity of pathologic specimens available for evaluation. Since then, several autopsy studies have demonstrated viral RNA in myocardial tissue, although it remains unknown if SARS-CoV-2 causes myocardial depression by direct myocyte invasion (Puelles et al. 2020).
Causes of Increased RV Afterload in the ICU
- Thrombosis: Acute pulmonary embolism can lead to an acute and severe decline in RV function. The diagnosis of pulmonary embolism should be considered in any patient with unexplained RV dysfunction. The risk of thrombosis and potential role for anti-thrombotic therapy in SARS-CoV-2 has been discussed in detail in prior FLAREs from March 31st, April 30th and May 8th
- Hypoxic Pulmonary Vasoconstriction: Alveolar hypoxia may occur in ICU patients, even in those without ARDS as their primary reason for ICU admission. Hypoxic pulmonary vasoconstriction was discussed in detail in the May 19th FLARE
- Acidosis: While the exact mechanisms are not completely understood, acidosis also raises the RV afterload. This also potentiates the pulmonary vascular response to hypoxia, so that the degree of increase in PVR at a given level of hypoxemia rises as acidosis worsens (Figure 6) (Ventetuolo and Klinger 2014)
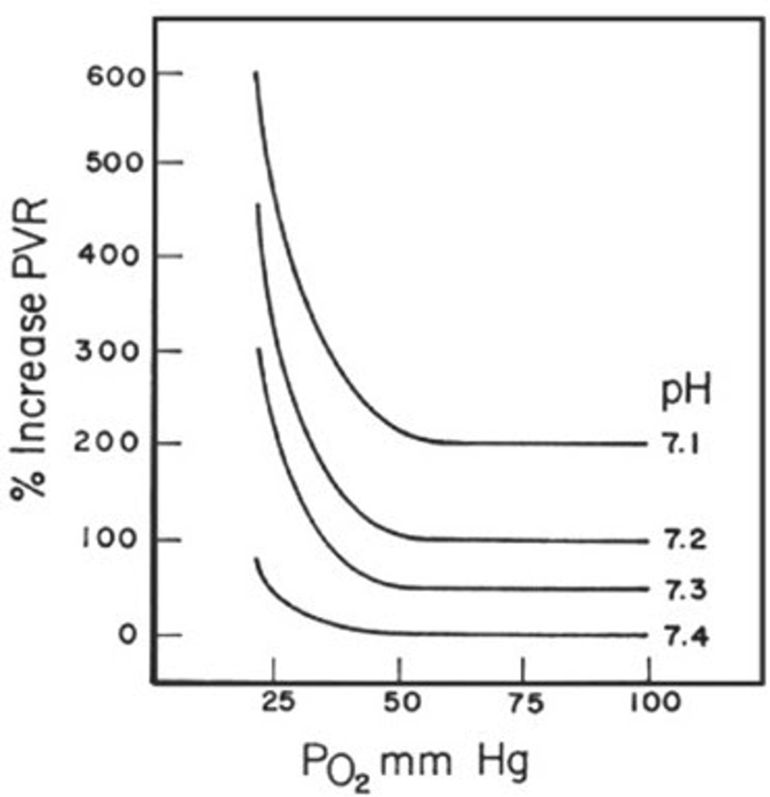
Figure 6
Changes in PVR as related to PO2 at different blood pH (Ventetuolo and Klinger 2014).
- Ventilation at high lung volume: As the pulmonary vasculature is embedded within the pulmonary parenchyma, the caliber of pulmonary vessels, and therefore the pulmonary vascular resistance, is affected by lung volume (Howell et al. 1961). The intra-alveolar capillaries are exposed to alveolar pressure while extra-alveolar vessels are tethered to the surrounding interstitium. As such, these vessels are variably stretched open or compressed depending on the lung volume. Extra-alveolar vessels have the lowest resistance at high lung volumes, at which tissue tethering is maximal. Conversely, intra-alveolar vessels have the lowest resistance at residual volume, at which the vessels are only minimally distorted by alveolar inflation. The net effect of these opposing trends is that the pulmonary vascular resistance is lowest at functional residual capacity (FRC) (Figure 7)
- Ventilation at high airway pressures: With severe lung injury and positive pressure ventilation, alveolar pressures can be high enough to exceed vascular pressures. This increase in alveolar pressure relative to vascular pressures can result in creation of dead space (ventilated but not perfused lung units), decreasing cross-sectional pulmonary vascular area and increasing resistance. Additionally, in lung units in which alveolar pressure is greater than pulmonary venous but not pulmonary arterial pressure, the alveolar pressure becomes the “downstream” pressure for the RV, and RV afterload is therefore directly increased by increases in alveolar pressure
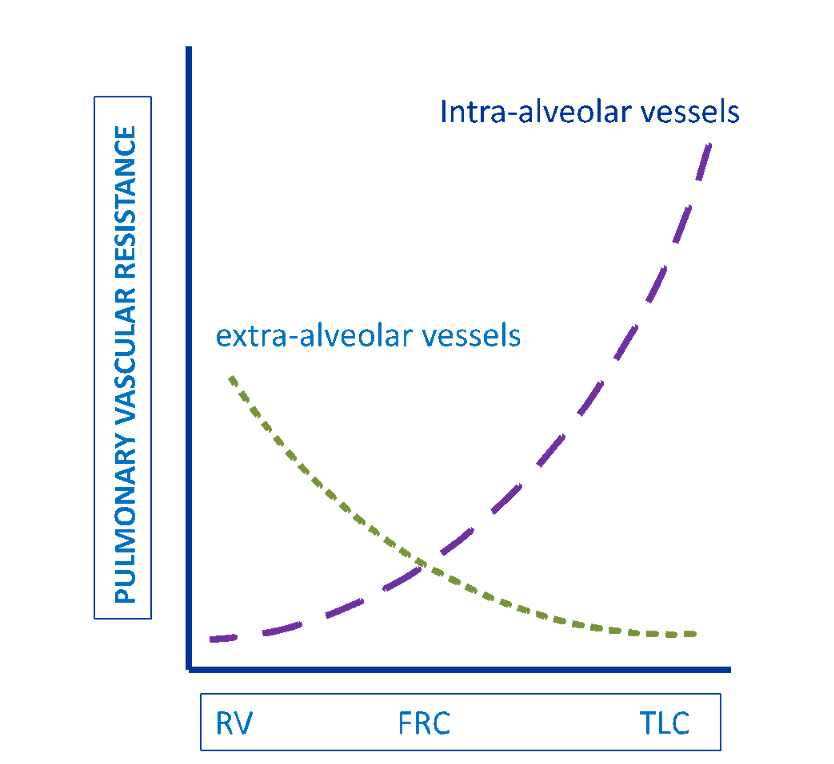
Figure 7
Pulmonary vascular resistance as related to lung volume (Ventetuolo and Klinger 2014).
Diagnosis of RV Dysfunction
The diagnosis of RV dysfunction is typically made via echocardiogram, which allows for both functional and anatomic assessment. Another useful test is CT scan, which not only provides information about the etiology of RV dysfunction (by identifying pulmonary emboli or significant parenchymal lung disease) but also identifies RV enlargement or septal flattening, with reasonable correlation with echocardiogram findings. These findings have prognostic significance in patients with acute RV failure due to pulmonary embolism (Aribas et al. 2014; Park et al. 2012).
Infection control concerns may limit the ability to obtain a full transthoracic echocardiogram or CT scan in a patient with SARS-CoV-2. Diagnosis can, however, be made by other means. Point-of-care ultrasound may allow for rapid bedside assessment. EKG findings such as complete or incomplete right bundle branch blocks, T-wave inversions in V1-V4 or the S1Q3T3 pattern should also raise concern for RV dysfunction (Park et al. 2012).
The importance of clinical and laboratory assessment should also not be underestimated. Physical exam findings suggesting significant RV dysfunction include elevated jugular venous pressure (a central venous pressure measurement can be used in patients with a central venous catheter), cool extremities, peripheral edema, a prominent P2, RV heave and/or a pulsatile liver. Laboratory findings may include elevations in NT-proBNP and evidence of end-organ dysfunction. In the ICU, hemodynamic variables such as central venous pressure (CVP) and central or mixed-venous oxygen saturation may be readily available.
Management of RV Failure
The detailed management of RV failure in the ICU is complex and outside the scope of this FLARE. However, we will discuss the general principles. Figure 8 provides a framework to think about interventions for a patient in RV failure.
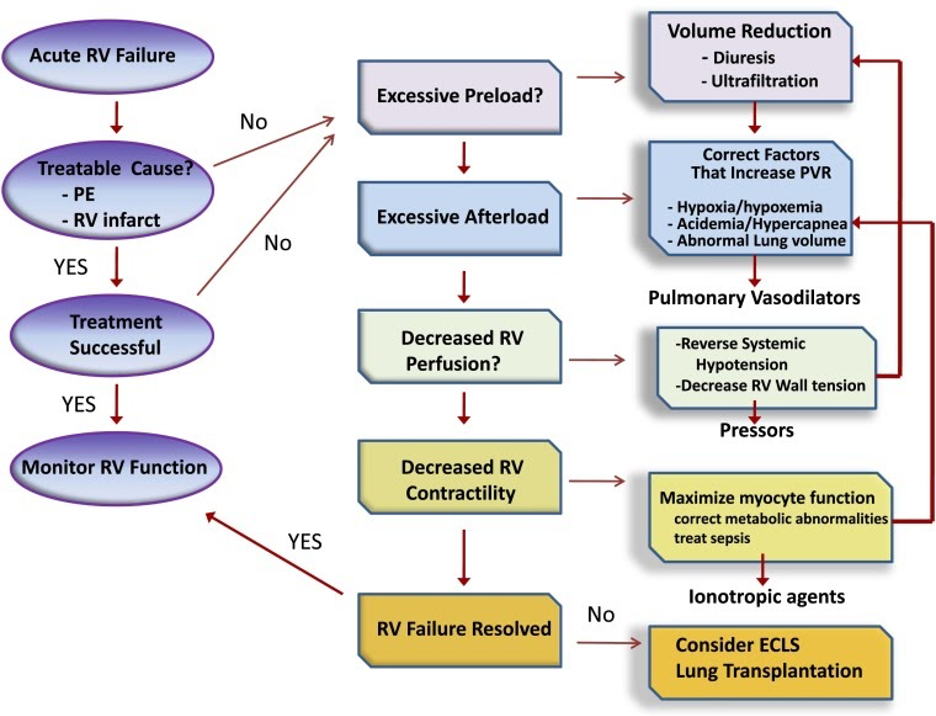
Figure 8
Approach to management of acute right ventricular (RV) failure. Patients should be assessed for acute causes of increased RV afterload or decreased contractility, such as pulmonary embolism or right-sided infarction. If no readily reversible cause is identified, efforts should be directed at optimizing RV preload and reducing RV afterload. The latter should include reversal of factors known to increase pulmonary vascular resistance and then the use of selective pulmonary vasodilator drugs. Metabolic conditions that reduce cardiac contractility, such as sepsis and acidemia, should be addressed. Systemic arterial pressure should be kept above RV systolic pressure to maintain RV perfusion. If these efforts fail, inotropic agents can be tried to improve RV contractility. Measures to correct metabolic abnormalities and reduce RV dilation will also aid RV contractility. Extracorporeal life support should be considered when medical therapy is unsuccessful in a patient who has a reversible cause of RV failure or who is being prepared for lung transplantation. ECLS = extracorporeal life support; PE = pulmonary embolism; PVR = pulmonary vascular resistance (Ventetuolo and Klinger 2014).
When possible, the underlying cause of the RV failure should be treated. For example, a patient with a PE may be amenable to thrombolysis or thrombectomy. In many situations, unfortunately, the cause of the RV failure may not be readily reversible, as is frequent in patients with ARDS.
When the underlying cause is not immediately reversible, management focuses on optimizing RV afterload and supporting the patient hemodynamically. Elevated preload can worsen RV dilation and ischemia, excess intravascular volume should be removed either via diuresis or with renal replacement therapy if significant renal dysfunction is present.
Efforts should be made to minimize the PVR. This includes correcting acidosis and hypoxia and ensuring the patient is not being ventilated at extremes of lung volume and pressure. Inhaled pulmonary vasodilators (nebulized epoprostenol or nitric oxide) can also be used to try to lower PVR. For patients with SARS-CoV-2, the risk of aerosolization with nebulized epoprostenol must be considered; inhaled nitric oxide may be preferred if available. Oral and parenteral pulmonary vasodilators are typically avoided in the acute setting given risks of systemic hypotension. Indeed, the systemic blood pressure should be supported with vasopressors as needed. Inotropes may be added to try to further support cardiac function. Finally, for appropriate patients, extracorporeal life support (ECLS or ECMO) may be considered.
RV Dysfunction in SARS-CoV-2
Two recent publications highlighted the potential for RV dysfunction in the setting of SARS-CoV-2 infection. Creel-Bulos et al. describe the finding of acute right ventricular failure in five patients with SARS-CoV-2 infection admitted to the ICU (Creel-Bulos et al. 2020). All patients had evidence of significant RV dysfunction by echocardiogram, 4 of 5 had hemodynamic instability and 3 of 5 died. Two patients had clot noted in the RV, and while it does not appear that all patients received a formal assessment for pulmonary embolism (PE), the authors postulate that the most likely explanation for the clinical scenario was acute pulmonary embolism (Creel-Bulos et al. 2020).
There are several limitations to this case series limiting our ability to draw broader conclusions. First, as not all patients received evaluation for PE and so it is impossible to know if the echocardiographic findings can be attributed to PE or if another etiology needs to be invoked. Additionally, as discussed above, RV dysfunction is common in patients with ARDS and sepsis and there is minimal information about the presence of hypoxemic respiratory failure or infection. Finally, there is no denominator of other critically ill patients and so it is unknown what proportion of ICU patients are represented by this case series. Without knowing what proportion of the patients with SARS-CoV-2 developed acute RV failure, there is no way to know if this is a complication occurring more commonly in COVID-19 patients.
Li et al. examined whether right ventricular longitudinal strain (RVLS) is associated with increased mortality in COVID-19. RVLS was measured by averaging the calculated strain over multiple segments of the RV free wall. They analyzed 120 consecutive patients with SARS-CoV-2 who underwent echocardiogram. A lower RVLS was associated with an increased risk of mortality as were other assessments of RV impairment including RV fractional area change (RVFAC) and tricuspid annular plane systolic excursion (TAPSE). The patients with the lowest RVLS were also more likely to have ARDS and require high flow oxygen and mechanical ventilation suggesting that the echocardiographic abnormalities may be a marker of disease severity rather than the primary driver of the observed increased mortality (Li et al. 2020). This study is consistent with prior studies showing an association with RV dysfunction and mortality, and the results are not unexpected.
Summary
Based on prior studies examining the incidence of RV dysfunction in patients with ARDS and shock, it is not surprising that some of patients with SARS-CoV-2 admitted to the ICU develop RV dysfunction. To date, there is no high quality data to indicate that patients with SARS-CoV-2 have a higher risk of RV dysfunction than would be expected based on their underlying ARDS. It is unlikely that the reports of RV dysfunction in this setting represent a novel phenomenon that is unique to SARS-CoV-2. Assessment and management of patients with RV dysfunction should follow usual ICU procedures and protocols.
References:
- Aribas, Alpay, Suat Keskin, Hakan Akilli, Mehmet Kayrak, Halil Ibrahim Erdogan, Ibrahim Guler, Oguzhan Yildirim, and Taha Tahir Bekci. 2014. “The Use of Axial Diameters and CT Obstruction Scores for Determining Echocardiographic Right Ventricular Dysfunction in Patients with Acute Pulmonary Embolism.” Japanese Journal of Radiology 32 (8): 451–60.
- Boissier, Florence, Sandrine Katsahian, Keyvan Razazi, Arnaud W. Thille, Ferran Roche-Campo, Rusel Leon, Emmanuel Vivier, et al. 2013. “Prevalence and Prognosis of Cor Pulmonale during Protective Ventilation for Acute Respiratory Distress Syndrome.” Intensive Care Medicine 39 (10): 1725–33.
- Bowles, Neil E., Jiyuan Ni, Debra L. Kearney, Matthias Pauschinger, Heinz-Peter Schultheiss, Robert McCarthy, Joshua Hare, J. Timothy Bricker, Karla R. Bowles, and Jeffrey A. Towbin. 2003. “Detection of Viruses in Myocardial Tissues by Polymerase Chain Reaction. Evidence of Adenovirus as a Common Cause of Myocarditis in Children and Adults.” Journal of the American College of Cardiology 42 (3): 466–72.
- Bull, Todd M., Brendan Clark, Kim McFann, and Marc Moss. 2010. “Pulmonary Vascular Dysfunction Is Associated with Poor Outcomes in Patients with Acute Lung Injury.” American Journal of Respiratory and Critical Care Medicine. https://doi.org/10.1164/rccm.201002-0250oc.
- Chan, Chee, and James R. Klinger. 2015. “Sepsis and Pulmonary Arterial Hypertension in the ICU.” Advances in Pulmonary Hypertension 13 (4): 188–96.
- Chan, Chee M., and James R. Klinger. 2008. “The Right Ventricle in Sepsis.” Clinics in Chest Medicine 29 (4): 661–76, ix.
- Creel-Bulos, Christina, Maxwell Hockstein, Neha Amin, Samer Melhem, Alexander Truong, and Milad Sharifpour. 2020. “Acute Cor Pulmonale in Critically Ill Patients with Covid-19.” The New England Journal of Medicine 382 (21): e70.
- Howell, J. B., S. Permutt, D. F. Proctor, and R. L. Riley. 1961. “Effect of Inflation of the Lung on Different Parts of Pulmonary Vascular Bed.” Journal of Applied Physiology 16 (January): 71–76.
- Kadokami, T., C. F. McTiernan, T. Kubota, C. S. Frye, G. S. Bounoutas, P. D. Robbins, S. C. Watkins, and A. M. Feldman. 2001. “Effects of Soluble TNF Receptor Treatment on Lipopolysaccharide-Induced Myocardial Cytokine Expression.” American Journal of Physiology. Heart and Circulatory Physiology 280 (5): H2281–91.
- Lhéritier, Gwenaëlle, Annick Legras, Agnès Caille, Thierry Lherm, Armelle Mathonnet, Jean-Pierre Frat, Anne Courte, et al. 2013. “Prevalence and Prognostic Value of Acute Cor Pulmonale and Patent Foramen Ovale in Ventilated Patients with Early Acute Respiratory Distress Syndrome: A Multicenter Study.” Intensive Care Medicine 39 (10): 1734–42.
- Li, Yuman, He Li, Shuangshuang Zhu, Yuji Xie, Bin Wang, Lin He, Danqing Zhang, et al. 2020. “Prognostic Value of Right Ventricular Longitudinal Strain in Patients with COVID-19.” JACC: Cardiovascular Imaging, April. https://doi.org/10.1016/j.jcmg.2020.04.014.
- McIntyre, K. M., and A. A. Sasahara. 1971. “The Hemodynamic Response to Pulmonary Embolism in Patients without Prior Cardiopulmonary Disease.” The American Journal of Cardiology 28 (3): 288–94.
- Mekontso Dessap, Armand, Florence Boissier, Cyril Charron, Emmanuelle Bégot, Xavier Repessé, Annick Legras, Christian Brun-Buisson, Philippe Vignon, and Antoine Vieillard-Baron. 2016. “Acute Cor Pulmonale during Protective Ventilation for Acute Respiratory Distress Syndrome: Prevalence, Predictors, and Clinical Impact.” Intensive Care Medicine 42 (5): 862–70.
- Naeije, Robert, and N. Chesler. 2012. “Pulmonary Circulation at Exercise.” Comprehensive Physiology 2 (1): 711–41.
- Park, Jeong Rang, Sung-A Chang, Shin Yi Jang, Hye Jin No, Sung-Ji Park, Seung-Hyuk Choi, Seung Woo Park, et al. 2012. “Evaluation of Right Ventricular Dysfunction and Prediction of Clinical Outcomes in Acute Pulmonary Embolism by Chest Computed Tomography: Comparisons with Echocardiography.” The International Journal of Cardiovascular Imaging 28 (4): 979–87.
- Price, Laura C., Stephen J. Wort, Simon J. Finney, Philip S. Marino, and Stephen J. Brett. 2010. “Pulmonary Vascular and Right Ventricular Dysfunction in Adult Critical Care: Current and Emerging Options for Management: A Systematic Literature Review.” Critical Care / the Society of Critical Care Medicine 14 (5): R169.
- Puelles, Victor G., Marc Lütgehetmann, Maja T. Lindenmeyer, Jan P. Sperhake, Milagros N. Wong, Lena Allweiss, Silvia Chilla, et al. 2020. “Multiorgan and Renal Tropism of SARS-CoV-2.” New England Journal of Medicine. https://doi.org/10.1056/nejmc2011400.
- Sutton, G. C., R. J. Hall, and I. H. Kerr. 1977. “Clinical Course and Late Prognosis of Treated Subacute Massive, Acute Minor, and Chronic Pulmonary Thromboembolism.” British Heart Journal 39 (10): 1135–42.
- Ventetuolo, Corey E., and James R. Klinger. 2014. “Management of Acute Right Ventricular Failure in the Intensive Care Unit.” Annals of the American Thoracic Society 11 (5): 811–22.
- Vieillard-Baron, Antoine, R. Naeije, F. Haddad, H. J. Bogaard, T. M. Bull, N. Fletcher, T. Lahm, et al. 2018. “Diagnostic Workup, Etiologies and Management of Acute Right Ventricle Failure : A State-of-the-Art Paper.” Intensive Care Medicine 44 (6): 774–90.
- Vlahakes, G. J., K. Turley, and J. I. Hoffman. 1981. “The Pathophysiology of Failure in Acute Right Ventricular Hypertension: Hemodynamic and Biochemical Correlations.” Circulation 63 (1): 87–95.
- Wolferen, Serge A. van, J. Tim Marcus, Nico Westerhof, Marieke D. Spreeuwenberg, Koen M. J. Marques, Jean G. F. Bronzwaer, Ivo R. Henkens, et al. 2008. “Right Coronary Artery Flow Impairment in Patients with Pulmonary Hypertension.” European Heart Journal 29 (1): 120–27.
- Zochios, Vasileios, Ken Parhar, William Tunnicliffe, Andrew Roscoe, and Fang Gao. 2017. “The Right Ventricle in ARDS.” Chest 152 (1): 181–93.
Learn more about research in the Division of Pulmonary and Critical Care Medicine
View all COVID-19 Updates